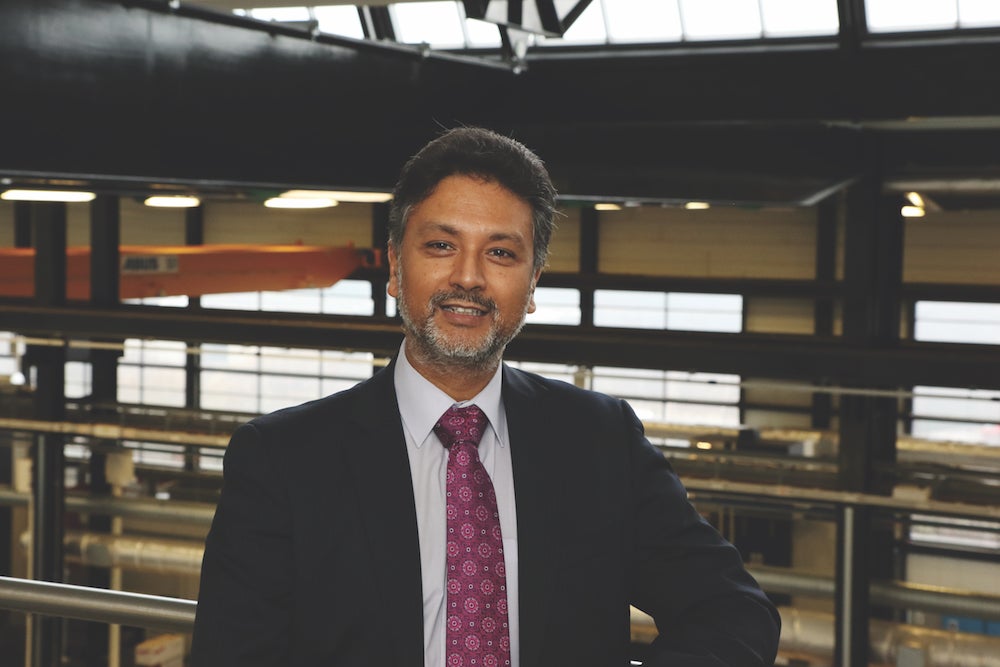
The UK is opening a new facility to develop and test materials and manufacturing processes in its quest to build the world’s first commercial nuclear fusion power station. Steve Jones, chief technology officer at the Nuclear AMRC, and professor of Welding technology at the University of Sheffield examines the scale of the challenge.
If nuclear fusion is to be a major contributor to meeting the UK’s target of net-zero greenhouse gas emissions from 2050, there is a need to combine advanced technologies with novel manufacturing philosophies to make it a commercial proposition.
We need to develop solutions that balance technical and cost benefits, ensure the supply chain has the right capabilities and deploy cost-effective manufacturing methods and mature processing technologies.
These nuclear fusion manufacturing challenges should not be underestimated. Consider the variety and extent of practices being deployed to deliver the international nuclear fusion project, Iter, under construction in France.
Such massively complex programmes have to be broken down into manageable modules and work packages to maximise engagement with the supply chain and ensure cost-effective delivery to schedule. Developing a modularisation philosophy to meet the needs of fusion will be high on the manufacturing agenda.
The Nuclear Advanced Manufacturing Research Centre (Nuclear AMRC), is working with the UK Atomic Energy Authority (UKAEA) on a feasibility study of modular construction techniques for nuclear fusion.
This work is part of the design development of the Spherical Tokamak for Energy Production (STEP), the UK’s bid to build the world’s first commercial fusion power station.
The study is investigating how the modularisation techniques being developed for small and advanced modular reactor designs could be applied to the STEP programme.
This research draws on our work to develop a through-life modularisation product structure. This is a systematic approach which can be used in the early design process for complex assemblies, to consider factors such as design style, module boundaries, interfacing methods and the degree of modularity required. The aim is to reduce risk in manufacturing and construction of new nuclear projects, and enable delivery of a modular system to schedule and budget that can be readily maintained throughout its service life, including decommissioning.
Finding the right materials for nuclear fusion
Developing comprehensive data about the array of materials which will be needed to build a commercial nuclear fusion reactor is a big engineering challenges.
Critical components such as first wall lining and divertors have to perform under extreme conditions of heat flux, vacuum and magnetic fields. Fabricating these components from exotic materials will require a full assessment of appropriate technologies and skills.
Suitable materials for these critical components will come from the class of refractory metals, with melting temperatures above 2000°C (3632°F). These include niobium, molybdenum, tantalum, tungsten, rhenium and their alloys.
For nuclear fusion, we must identify metals which can avoid problematic radioactive isotope formation, resist ion bombardment, avoid embrittlement and have good thermomechanical stability with limited tritium sequestration. This considerably narrows the choice of material.
We also have to consider other advanced materials such as ceramic-metal composites and other ceramic composites. Qualifying these for safety-critical nuclear fusion applications will require significant advances in integrity assessment techniques, such as the use of graphene sensors. These have been proposed as a tool for defect detection and predictive maintenance in composite structures.
Tackling these challenges will be the job of UKAEA’s new material testing facility, under construction at the Advanced Manufacturing Park in Yorkshire, also home to the Nuclear AMRC’s research factory.
When it opens in autumn 2020, the facility will develop and test materials and components for nuclear fusion, including novel metals and ceramics, under simulated reactor conditions.
Joining techniques for materials used in nuclear fusion projects

Much of the research at the UKAEA facility will focus on joining techniques for fusion materials.
We are seeing a lot of interest in advanced power-beam welding processes, including electron beam and laser technologies, with integral inspection systems. These can produce and inspect autogenous and heterogeneous welds with reduced cracking susceptibility, residual stress and distortion of complex geometries while simultaneously determining the joint’s integrity.
Some of these power beam techniques, including electron beam welding, require a vacuum so are currently deployed within a vacuum chamber. There is a lot of interest in local vacuum techniques, which allow single-pass welds of thick sections without needing a vacuum chamber large enough to contain the entire assembly.
The Nuclear AMRC has worked with partners including TWI, Wood and Cammell Laird on local-vacuum electron beam welding techniques, in projects funded by the UK government through the Nuclear Innovation Programme.
Fusion components will also require dissimilar metals and materials to be joined, using solid-state bonding techniques such as diffusion bonding, ultrasonic bonding, electromagnetic pulse bonding and hot isostatic pressing.
It is critical to be able to machine such materials. This will rely on specialised techniques, such as supercritical carbon dioxide cooling, to support tool life and metal removal rates. The Nuclear AMRC is already working on these techniques, but developments in machining technology require further study to improve confidence in product integrity and assess the best fabrication solution.
Additive manufacturing and its role in nuclear fusion
As an alternative to traditional fabrication and subtractive machining, there is interest in additive manufacturing and other near-net shape forming techniques for nuclear fusion.
Direct energy deposition techniques combine welding tools with automated control to accurately deposit wire or powder. They are already used in aerospace and other industries, but need further development for nuclear fusion applications.
Early research has shown that depositing tungsten and niobium in their pure form using wire and powder within high-quality inert atmospheres can still give rise to problems such as through-thickness cracking and internal microfissures.
Near-net shaped forming, exploiting superplastic and solid-state techniques, should also be explored further. These can produce dissimilar metal bonds and functionally graded components with maximised inherent thermophysical properties to supplement the part’s performance conditioning. It may be possible to tailor their chemistry to improve fabrication requirements such as stiffness and inspectability.

Advanced numerical simulation to predict component performance
Advanced numerical simulation techniques will allow designers to push the envelope on component performance. That is likely to include predictive analytics that use various self-diagnostic neural network algorithms.
We should also develop tactile and remote in-process inspection methods for nuclear fusion, using ultrasonics, vision systems, advanced electromagnetic acoustic transducers and airborne acoustic system analysis. These should be coupled with advances in deep learning artificial neural networks which will need to be embedded seamlessly within a production schedule.
Codes and standards needed to make fusion power a commercial reality
Bringing fusion power from the research world to commercial engineering reality will require a host of advanced manufacturing technologies and, no less vitally, suitable design and manufacturing codes.
The Iter programme has adopted the well-established France AFCEN codes (RCC-M, RCC-C, RCC-E, RCC-M and RCC-MRx), but manufacturing codes specific to nuclear fusion reactor technology have to be developed for commercial fusion projects. Such codes will only become effective once service data has been analysed.